Organ printing
A printable organ is an artificially constructed device designed for organ replacement, produced using 3D printing techniques. The primary use of printable organs is in transplantation. Research is currently being conducted on artificial heart, kidney, and liver structures, as well as other major organs. For more complicated organs, such as the heart, smaller constructs such as heart valves have also been the subject of research. Some printed organs are approaching functionality requirements for clinical implementation, and primarily include hollow structures such as the bladder, as well as vascular structures such as urine tubes.[1][2]
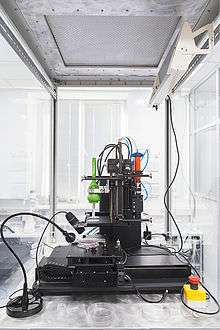
Modified inkjet printers have been used to produce three-dimensional biological tissue. Printer cartridges are filled with a suspension of living cells and a smart gel, the latter used for providing structure. Alternating patterns of the smart gel and living cells are printed using a standard printing nozzle, with cells eventually fusing together to form tissue. When completed, the gel is cooled and washed away, leaving behind only living cells.
History
3D printing for producing a cellular construct was first introduced in 2003, when Thomas Boland of Clemson University patented the use of inkjet printing for cells. This process utilized a modified spotting system for the deposition of cells into organized 3D matrices placed on a substrate.[3][4]
Since Boland's initial findings, the 3D printing of biological structures, also known as bioprinting, has been further developed to encompass the production of tissue and organ structures, as opposed to cell matrices. Additionally, more techniques for printing, such as extrusion bioprinting, have been researched and subsequently introduced as a means of production.[5]
Organ printing has been approached as a potential solution for the global shortage of donor organs. Organs that have been successfully printed and implemented in a clinical setting are either flat, such as skin, vascular, such as blood vessels, or hollow, such as the bladder. When artificial organs are prepared for transplantation, they are often produced with the recipient's own cells.
More complex organs, namely those that consist of solid cellular structures, are undergoing research; these organs include the heart, pancreas, and kidneys. Estimates for when such organs can be introduced as a viable medical treatment vary.[2] In 2013, the company Organovo produced a human liver using 3D bioprinting, though it is not suitable for transplantation, and has primarily been used as a medium for drug testing.[6] In 2018, doctors from Belfast transplanted a 3D-printed kidney replica.[7]
3D printing techniques
3D printing for the manufacturing of artificial organs has been a major topic of study in biological engineering. As the rapid manufacturing techniques entailed by 3D printing become increasingly efficient, their applicability in artificial organ synthesis has grown more evident. Some of the primary benefits of 3D printing lie in its capability of mass-producing scaffold structures, as well as the high degree of anatomical precision in scaffold products. This allows for the creation of constructs that more effectively resemble the microstructure of a natural organ or tissue structure.[8]
Organ printing using 3D printing can be conducted using a variety of techniques, each of which confers specific advantages that can be suited to particular types of organ production.
Drop-based bioprinting (Inkjet)
Drop-based bioprinting creates cellular constructions using individual droplets of a designated material, which has oftentimes been combined with a cell line. Upon contact with the substrate surface, each droplet begins to polymerize, forming a larger structure as individual droplets begin to coalesce. Polymerization is instigated by the presence of calcium ions on the substrate, which diffuse into the liquified bioink and allow for the formation of a solid gel. Drop-based bioprinting is commonly used due to its efficient speed, though this aspect makes it less suitable for more complicated organ structures.[9]
Extrusion bioprinting
Extrusion bioprinting involves the constant deposition of a particular printing material and cell line from an extruder, a type of mobile print head. This tends to be a more controlled and gentler process for material or cell deposition, and allows for greater cell densities to be used in the construction of 3D tissue or organ structures. However, such benefits are set back by the slower printing speeds entailed by this technique. Extrusion bioprinting is often coupled with UV light, which photopolymerizes the printed material to form a more stable, integrated construct.[5]
Printing materials
Materials for 3D printing usually consist of alginate or fibrin polymers that have been integrated with cellular adhesion molecules, which support the physical attachment of cells. Such polymers are specifically designed to maintain structural stability and be receptive to cellular integration. The term "bioink" has been used as a broad classification of materials that are compatible with 3D bioprinting.[10]
Printing materials must fit a broad spectrum of criteria, one of the foremost being biocompatibility. The resulting scaffolds formed by 3D printed materials should be physically and chemically appropriate for cell proliferation. Biodegradability is another important factor, and insures that the artificially formed structure can be broken down upon successful transplantation, to be replaced by a completely natural cellular structure. Due to the nature of 3D printing, materials used must be customizable and adaptable, being suited to wide array of cell types and structural conformations.[11]
Hydrogel alginates have emerged as one of the most commonly used materials in organ printing research, as they are highly customizable, and can be fine-tuned to simulate certain mechanical and biological properties characteristic of natural tissue. The ability of hydrogels to be tailored to specific needs allows them to be used as an adaptable scaffold material, that are suited for a variety of tissue or organ structures and physiological conditions.[5] A major challenge in the use of alginate is its stability and slow degradation, which makes it difficult for the artificial gel scaffolding to be broken down and replaced with the implanted cells' own extracellular matrix[12]. Alginate hydrogel that is suitable for extrusion printing is also often less structurally and mechanically sound; however, this issue can be mediated by the incorporation of other biopolymers, such as nanocellulose, to provide greater stability. The properties of the alginate or mixed-polymer bioink are tunable and can be altered for different applications and types of organs[12].
Cell sources
The creation of a complete organ often requires incorporation of a variety of different cell types, arranged in distinct and patterned ways. One advantage of 3D-printed organs, compared to traditional transplants, is the potential to use cells derived from the patient to make the new organ. This significantly decreases the likelihood of transplant rejection, and may remove the need for immunosuppressive drugs after transplant, which would reduce the health risks of transplants. However, since it may not always be possible to collect all the needed cell types, it may be necessary to collect adult stem cells or induce pluripotency in collected tissue[5]. This involves resource-intensive cell growth and differentiation and comes with its own set of potential health risks, since cell proliferation in a printed organ occurs outside the body and requires external application of growth factors. However, the ability of some tissues to self-organize into differentiated structures may provide a way to simultaneously construct the tissues and form distinct cell populations, improving the efficacy and functionality of organ printing[13].
References
- Berthiaume, François; Maguire, Timothy J.; Yarmush, Martin L. (2011). "Tissue Engineering and Regenerative Medicine: History, Progress, and Challenges". Annual Review of Chemical and Biomolecular Engineering. 2: 403–30. doi:10.1146/annurev-chembioeng-061010-114257. PMID 22432625.
- Cooper-White, Macrina. "How 3D Printing Could End The Deadly Shortage Of Donor Organs". Huffington Post. Retrieved 27 March 2015.
- Boland, Thomas. "Patent US7051654: Ink-jet printing of viable cells". Google.com. Retrieved 31 March 2015.
- Auger, François A.; Gibot, Laure; Lacroix, Dan (2013). "The Pivotal Role of Vascularization in Tissue Engineering". Annual Review of Biomedical Engineering. 15: 177–200. doi:10.1146/annurev-bioeng-071812-152428. PMID 23642245.
- Bajaj, Piyush; Schweller, Ryan M.; Khademhosseini, Ali; West, Jennifer L.; Bashir, Rashid (2014). "3D Biofabrication Strategies for Tissue Engineering and Regenerative Medicine". Annual Review of Biomedical Engineering. 16: 247–76. doi:10.1146/annurev-bioeng-071813-105155. PMC 4131759. PMID 24905875.
- Bort, Julie. "Biotech Firm: We Will 3D Print A Human Liver In 2014". Business Insider. Retrieved 1 April 2015.
- https://web.archive.org/web/20190418075242/https://www.sculpteo.com/blog/2018/05/31/3d-printed-kidney-what-is-actually-possible/
- Hockaday, L A; Kang, K H; Colangelo, N W; Cheung, P Y C; Duan, B; Malone, E; Wu, J; Girardi, L N; Bonassar, L J; Lipson, H; Chu, C C; Butcher, J T (2012). "Rapid 3D printing of anatomically accurate and mechanically heterogeneous aortic valve hydrogel scaffolds". Biofabrication. 4 (3): 035005. Bibcode:2012BioFa...4c5005H. doi:10.1088/1758-5082/4/3/035005. PMC 3676672. PMID 22914604.
- Auger, François A.; Gibot, Laure; Lacroix, Dan (2013). "The Pivotal Role of Vascularization in Tissue Engineering". Annual Review of Biomedical Engineering. 15: 177–200. doi:10.1146/annurev-bioeng-071812-152428. PMID 23642245.
- Kesti, Matti; Müller, Michael; Becher, Jana; Schnabelrauch, Matthias; d'Este, Matteo; Eglin, David; Zenobi-Wong, Marcy (2015). "A versatile bioink for three-dimensional printing of cellular scaffolds based on thermally and photo-triggered tandem gelation". Acta Biomaterialia. 11: 162–72. doi:10.1016/j.actbio.2014.09.033. hdl:20.500.11850/103400. PMID 25260606.
- Augst, Alexander D.; Kong, Hyun Joon; Mooney, David J. (2006). "Alginate Hydrogels as Biomaterials". Macromolecular Bioscience. 6 (8): 623–33. doi:10.1002/mabi.200600069. PMID 16881042.
- Axpe, Eneko; Oyen, Michelle L. (2016-11-25). "Applications of Alginate-Based Bioinks in 3D Bioprinting". International Journal of Molecular Sciences. 17 (12): 1976. doi:10.3390/ijms17121976. PMC 5187776. PMID 27898010.
- Athanasiou, Kyriacos A.; Eswaramoorthy, Rajalakshmanan; Hadidi, Pasha; Hu, Jerry C. (2013-07-11). "Self-Organization and the Self-Assembling Process in Tissue Engineering". Annual Review of Biomedical Engineering. 15 (1): 115–136. doi:10.1146/annurev-bioeng-071812-152423. ISSN 1523-9829. PMC 4420200. PMID 23701238.